Ernest Rabinowicz, retired professor emeritus at Massachusetts Institute of Technology (MIT), suggests that 70 percent of machines are removed from service due to degradation of mechanical surfaces.
Degradation may occur as a result of abrasive, adhesive, erosive, corrosive or fatigue-induced wear. A clear understanding of how these wear modes develop will assist the lubrication technician in understanding the importance of his/her role in improving machine reliability.
The author will address bearing and gearing surface degradation leading to pitting and spalling, and will address the nature of subsurface material degradation that causes spalling. The article will also introduce the influence of filtration and use of solid film additives to influence surface degradation and improve reliability.
Possible Bearing Failure Mode with Hard Particle Contamination
In elastohydrodynamic (EHD) lubrication regimes, it is statistically more difficult for contaminant particles to enter the contact patch because of the squeeze effect at nonconformal contacts. Also, the minimum film thicknesses tend to be many times smaller geometrically than for hydrodynamic lubrication films (typically 5 μm compared to 100 μm).
However, offsetting this somewhat is the phenomenon that the viscosity of lubricants is pressure-dependent. Increasing pressure increases viscosity. In typical rolling bearing contacts, the viscosity can increase a thousandfold for a pressure rise of 300 MPa (43,511 psi). This is quite possible for hertzian contacts.
The increased viscosity due to pressure has greater capacity to trap and hold contaminant particles in the contact region. For proper EHD lubrication of all hertzian contacts including rolling bearings, the film thickness must be approximately three times the combined surface roughness of the contacting parts to avoid asperity intersection and boundary lubrication.
Rolling bearings are manufactured with finely ground and polished surfaces so that the EHD film thicknesses will easily satisfy this requirement.
Bearing Failure Examination
Researchers (Loewenthal and Moyer 1979) conducted fatigue tests on groups of 65 mm bore diameter deep-groove ball bearings with a noncontaminated MIL-L-23699 lubricant and with a contaminated lubricant under four levels of filtration. Tests conducted with the noncontaminated lubricant provided baseline data. These tests used prefiltered oil in a recirculating lubrication system containing a 49 μm absolute (30 μm nominal) full-flow filter.
Table 1. Summary of Loewenthal and Moyer (1979) Experiments
In the remaining series of tests, contaminants of a composition similar to that found in filters from aircraft gas turbine engines (Table 1) were injected into the test filter’s supply line at a constant rate of 125 milligrams per hour per bearing. The test filters, of porous depth media construction, had absolute particle removal ratings of 3, 30, 49, and 105 μm (0.45, 10, 30 and 70 μm nominal), respectively.
The test conditions included a bearing shaft speed of 15,000 rpm, a radial load of 4580 N (1,030 pounds) producing a maximum Hertz stress of approximately 2,410 MPa (350,000 PSI) on the bearing inner race. The temperature of the lubricant into the test bearing and the pump temperature were maintained at 347°K (165°F). The results are plotted as distributions on a Weibull (log-log2) graph of cumulative failure versus time to failure (Figure 2).
Figure 2. Failure Distributions for Loewenthal
and Moyer (1979) Bearing Fatigue Life Experiments.
Table 1 summarizes the test results. Note that there were no fatigue failures with the coarse (105 μm) filter. A mixture of stainless steel sub 44 μm particles, AC a coarse test dust (ACCTD) and carbon-graphite test dust was used for the tests.
Figure 1. Typical Denting due to Trapping
Small-scale Contaminants in Rolling
Contacts (Scale Bar is 15 µm).
Figure 1 is a micrograph of a smooth bearing surface that still has machining marks but no evidence of scuffing wear. However, there are many surface defects due to contaminants being trapped in the EHD film. A, B and C are scattered contaminant indenting. D, E and F are microspalls that have initiated at dents. They are fatigue spall sites from which damage will progress.
Note that the shape factors (β= slope on the Weibull graph) have heightened with increase in filter coarseness. (For a brief explanation of the use of Weibull Statistics in reliability calculations, visit http://www.weibull.com/basics/lifedata.htm.)
Metallurgical examinations of the test bearing failures were made with the intent of determining the precise origin of the failure. In most cases, direct evidence of a surface defect-initiated spall was destroyed by spall propagation and/or camouflaged by secondary spall debris damage.
In several cases where the bearings were suspected of subsurface-initiated failure (subsurface cracking), metallographic cross-sections were made through the spall area. These examinations failed to uncover conditions such as faulty microstructure, nonmetallic inclusions or carbide agglomeration that would conclusively verify subsurface origin. Microscopic examination of the shape and depth of the spall generally gave the best indication of the most probable failure mode.
Many of the incipient spalls on failed bearings from the baseline, noncontaminated lubricant test series were elliptically shaped, relatively deep and steep sloped, giving the appearance of being subsurface-initiated. Examination of the spalls from the other test series with a contaminated lubricant revealed that fatigue failures were both surface- and subsurface-initiated with an increase in surface-initiated spalls coincidental with an increase in filter element particle pore size.
Also the mechanism of surface spall initiation appeared to shift with filter size. Point surface originated spalls, characterized by a shallow, arrowhead pattern appeared to be the most prevalent in the case of the 3 μm absolute filter tests. Micropitting, the result of debris particles interrupting the elastohydrodynamic film, was the most prevalent in tests with 49 μm filtration as described earlier.
Debris dents significantly larger than the absolute pore size of the filter were occasionally found on suspended (clean oil) test bearings, indicating that the debris particles can be generated within the bearing assembly itself. However, the number of these particles and their potential to initiate fatigue is judged relatively small in comparison to debris ingested externally or generated within the lubrication system and not removed by the filter. Therefore, to provide maximum component life, a concerted effort must be made to prevent contaminants from gaining entry into the system through proper sealing, and to remove contaminants through proper filtration.
The following results were obtained:
-
Bearing running track condition and fatigue life, to a lesser extent, generally improved with finer filtration. Tests with the baseline noncontaminated lubricant bearings produced the longest fatigue lives. Differences in the 10 percent lives of these bearings and those in contaminated lubricant tests with 3 μm and 30 μm absolute filtration were statistically insignificant, but were statistically significant for the bearing tested with a 49 μm absolute filter.
-
Surface distress and wear of the test bearings’ running track markedly increased with coarser filter size. Gross wear of bearings tested with a 105 μm absolute (70 μm nominal) filter precluded the onset of rolling element fatigue.
-
The bearing life dispersion parameter or Weibull slope increased with coarser filtration.
-
Fatigue failures were both surface- and subsurface-initiated with a trend toward more surface-initiated failures with coarser filtration. All of the failed bearings tested with 49 μm absolute filtration in a contaminated lubricant exhibited evidence of micropitting and surface-initiated failure.
Contamination
Most of the different contaminants that can be ingested or generated in a machine can have serious effects on the life of rolling bearings. However, the most common are ingested dusts (such as SiO2). Certain sizes of these hard abrasive particles can enter the contact zone and cause extreme impact pressures by bridging across the gap and removing the EHD film.
Scuffing, abrasive wear, polishing by the smaller particles and indentation by the larger particles are among the main causes for premature demise of the bearings. Loewenthal and Moyer (1979) made an experimental study of fatigue life of deep groove ball bearings for various filter sizes in a contaminated lubricant.
Polishing may lead to excessive clearances, impacts and early degradation. The jamming action of hard particles in the contact zone may cause ligament collapse (fracture of the remaining material between two cracks or a crack and the surface in a few load cycles – low cycle fatigue) at depths, which could be quite different from the normal high-cycle fatigue phenomenon.
The indentations themselves could become stress concentration sites and accelerate the deterioration and progression of low-cycle fatigue. Once fatigue has initiated in rolling bearings, it usually worsens at an increasing rate because the particles that spall out from the surface represent more particles that can get jammed in the contact zone and the effects grow exponentially.
Effects of Moly on Rolling Bearings
Experimental evidence (Kuhnell and Stecki, 1988) on two populations of 60 bearings with and without molybdenum disulfide (MoS2) yielded Β values of 2.4 and 1.6 respectively. The tests were carried out with identical conditions, circulating oil and full flow filtration of 3 μm. In the latter case, this meant that the longest lasting bearing survived for 20 times the life of the first to fail. Failure was indicated by the appearance of fatigue wear particles in the oil.
The characteristic life η can be chosen arbitrarily but common values are 10 percent (the life at which 10 percent of the population has failed, as the bearing companies use for their catalog dynamic load capacity figures), 50 percent (a median life) or 63.8 percent (which corresponds to the time constant of a first-order system response).
Incidentally, the characteristic life (time at which 63.8 percent had failed) of the bearings with MoS2 was 60 percent greater than that for the same oil without MoS2 with a 95 percent confidence level.
MoS2 is used as a solid lubricant because of its unique properties. It has a hexagonal lamellar structure with molybdenum and sulfur atoms arranged in parallel planes. The bonds on these planes are weak. They allow easy cleavage at intervals of approximately 0.5 μm with a Mohs’ hardness number between 1 and 2 (between those of talc and gypsum).
Materials with Mohs’ hardness number of 1 leave a mark when rubbed on paper. However, MoS2 is anisotropic and has great strength perpendicular to the slippage planes. In that direction, the Mohs’ hardness number is close to 8. This is harder than quartz (with a Mohs’ hardness number of 7). Therefore it has a high supporting strength combined with easy shear. The MoS2 plates out on surfaces to provide heavy-duty assistance to lubrication.
MoS2 is mined from deposits in their natural state as a mixture of many minerals and sand (SiO2). Extensive and expensive refining is necessary to extract the pure MoS2 needed to form lubricant additives. The Kuhnell and Stecki (1988) studies described above used MoS2 of ultra purity (less than 0.02 percent SiO2) and less than 3 μm size. The experiments of Ishabashi with negative results, used MoS2 from a different source and there was no information given as to its purity.
It is known that some commercially available MoS2 is supplied with 0.2 to 0.4 percent (SiO2) which is an abrasive. Given the apparently opposite results, it was surmised that the tests, which indicated that MoS2 reduced fatigue life, were possibly performed with additives having higher SiO2 content.
Tests conducted with noncontaminated oil and MoS2 as described above (Kuhnell and Stecki, 1988) revealed that the bearings could be run for some 125 percent to 150 percent of the time taken for initial spalling to occur before being in danger of collapse. In other words, the earlier the onset of fatigue damage, the more rapid the rate of deterioration.
However, in real applications the noise levels of the damaged bearings would not normally be tolerated and the bearings would have been removed earlier.
A plausible explanation could be that the time to fatigue initiation is a function of the number of potential fatigue sites (subsurface defects). If there are more such sites, there is more likelihood of triggering the fatigue process earlier and because there are more sites, the progression would be at a faster rate.
It is not clear if this same phenomenon (faster deterioration with earlier fatigue onset) would apply if the fatigue is initiated by contaminants. However, if the contamination is able to generate fatigue sites at indentations, then the same argument could apply.
Concluding Remarks
There are still many unknown factors concerning the physical mechanism of surface damage phenomena. The theoretical studies outlined above offer plausible explanations for nonconformal contact fatigue spalling under clean conditions and based on two-dimensional analyses. The effects of lubrication were not directly included. Extension of the work to three-dimensional analyses and inclusion of lubricant effects still need to be addressed. Nevertheless, the theories are compatible with experimental work both in-house and by others.
Weibull Distribution of Rolling Bearing Failure
Rolling element bearings are generally found to have a Weibull probability distribution function (PDF) of age-related (high cycle fatigue) failures. This distribution is common for mechanical devices, which are typically assemblages of numbers of machine elements, each of which individually may have other failure PDFs. The Weibull PDF is given by:
f(t)= | β (t-t0)β-1 | e-(t-to)β |
n | (n) | (n) |
where: f(t) = failure rate at time t
β= shape factor
t = characteristic life
t0 = minimum life
Because of the form of the Weibull PDF:
f(t)=Ce-tβ
if β is less than 1.0 the failure rate f(t) reduces;
if β is equal to 1.0 the failure rate f(t) is constant;
if β is greater than 1.0 the failure rate f(t) increases.
Editor’s Note
A version of this article was first published in “Systems Integrity and Maintenance,” the proceedings of the Third Asia Pacific Conference on Systems Integrity and Maintenance; Cairns, Australia, September 2002.
References
-
Rabinowicz, E. Friction and Wear of Materials, 2 nd ed. John Wiley and Sons: New York. 1995.
-
Ding, Y. and Kuhnell, B. “The Physical Cause of Spalling in Gears.” Machine Condition Monitoring,The Research Bulletin of the Centre for Machine Condition Monitoring. Vol. 9. Monash University. 1997.
-
Kuhnell, B. and Stecki, J. “Effects of MoS 2 on the Life of Heavily Loaded Rolling Element Bearings.” Tribology Transactions: Journal of the STLE, Vol. 31, 3. p. 325-328. July 1988.
-
Popinceanu, N., Galitanu, M., Cretu, S., Dianescu, E. and Hostuic, L. “Rolling Bearing Fatigue Life and E.H.L. Theory.” Wear 45, p. 17-32. 1977.
-
Ishibashi, A., Hoyashita, S. and Nakajima, A. “Effects of MoS 2 Particles in Oil on the Pitting of Rolling/Sliding Contact Surfaces Under Nearly Full EHL Conditions.” Proceedings of the 5th Leeds-Lyon-Symposium on Tribology, p. 282-290. Inst. of Mech. Eng.: London. 1979.
-
Dowson, D. and Higginson, G. Elastohydrodynamic Lubrication, SI Edition. Pergamon Press: U.K. 1977.
-
Loewenthal, S. and Moyer, D. “Filtration Effects on Ball Bearing Life and Condition in a Contaminated Lubricant.” Journal of Lubrication Technology, Transactionsof the ASME, Vol. 101, p. 171-179. April 1979.
-
Tallian, T. “Prediction of Rolling Contact Fatigue Life in Contaminated Lubricant: Part II – Mathematical Model.” Journal of Lubrication Technology, Transactions of the ASME, Vol. 98, No. 2. p. 251–257. 1976.
-
Tallian, T. “Prediction of Rolling Contact Fatigue Life in Contaminated Lubricant: Part II - Experimental.” Journal of Lubrication Technology, Transactions of the ASME, Vol. 98, No. 3, p. 384–392. 1976.
-
Ding, Y., Kuhnell, B. and Jones, R. “An Experimental Investigation of Gear Spalling Phenomenon.” Surface Modification Technologies X, Edited by T. Sudarshan, K. Khor and M. Jeandin. p. 63-74. The Institute of Materials: London. 1997.
-
Tallian, T. Failure Atlas for Hertz Contact Machine Elements, New York, New York. 1992.
-
Alban, L. Systematic Analysis of Gear Failures. ASM International: Metals Park, Ohio. 1985.
-
Way, S. “Pitting Due to Rolling Contact.” Journal of Applied Mechanics, Transactions of the ASME, Vol. 2, A49. 1935.
-
Keer, L. and Bryant, M. “A Pitting Model for Rolling Contact Fatigue.” Journal of Lubrication Technology. Transactions of the ASME, Vol. 105, p. 198-205. April 1983.
-
Bower, A. “The Influence of Crack Face Friction and Trapped Fluid on Surface-initiated Rolling Contact Fatigue Cracks.” Transactions of the ASME, Journal of Technology, Vol. 110, p. 704-711. 1988.
-
Cheng, H., Keer, L. and Mura, T. “Analytical Modelling of Surface Pitting in Simulated Gear Teeth Contacts,” SAE Technical Paper No. 841086, p. 4.987-4.995. 1984.
-
Fleming, J. and Suh, N. “Mechanics of Crack Propagation in Delamination Wear.” Wear 44, p. 39-56. 1977.
-
Kaneta, K., Murakami, Y. and Okazaki, T. “Growth Mechanism of Subsurface Crack Due to Hertzian Contact.” Journal of Tribology, Transactions of the ASME, Vol. 108, p. 134-139. 1986.
-
Sin, H. and Suh, N. “Subsurface Crack Propagation Due to Surface Traction in Sliding Wear.” Journal of Applied Mechanics, Transactions of the ASME, Vol. 51, p. 317-323. June 1984.
-
Ding, Y., Jones, R. and Kuhnell, B. “Numerical Analysis of Subsurface Crack Failure Beneath the Pitch Line of a Gear Tooth During Engagement.” Wear 185, p. 141-149. 1995.
-
Ding, Y., Jones, R. and Kuhnell B. “Elastic-plastic Finite Element Analysis of Spall Formation in Gears.” Wear 197, p. 197-205. 1996.
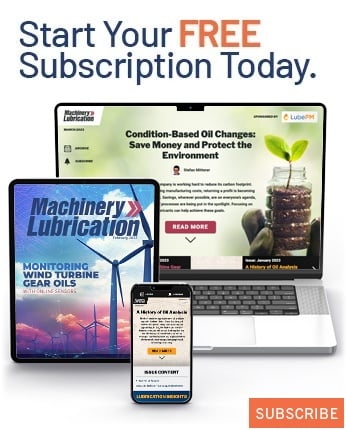